EVs and battery storage capacity will lead the demand for critical minerals due to their growing importance in curbing global greenhouse gas emissions. Lithium, nickel, cobalt, copper, graphite, manganese, and Rare Earth Elements (REE) will be crucial elements in the production of EVs and batteries. It is worth looking at some of the challenges that are expected in the supply chain of each respective mineral. This will help to put the more general numbers on supply and demand in a broader context. A mine may seem to have sufficient supply for the next decade, but it might not be the sort of supply that battery producers are looking for, still posing a risk to the supply chain of clean energy solutions.
Lithium
Demand for lithium-ion batteries (LiBs) is projected to grow at a compounded annual growth rate (CAGR) of 33% to reach just over 1 TWh by 2025, with the larger part of the demand growth coming from batteries for EVs as illustrated in Table 1 below.1 1 TWh in battery capacity demand requires between 700,000 and 800,000 t of lithium carbonate equivalent (LCE).2 Global production of LCE in 2020 was about 350,000 t meaning that in the next four years, production needs to double in order to meet the global demand in LiBs. A colossal challenge, especially for Europe, given that it does not produce any LCE on a commercial scale today.
With 500 Gwh of battery capacity set as a target for 2030, Europe will need to secure around 400,000 t of LCEs per year.3 These LCEs need to be processed, upgraded, or converted to battery grade LCEs before they can be used in the chemistries required for a LiB’s cathode.
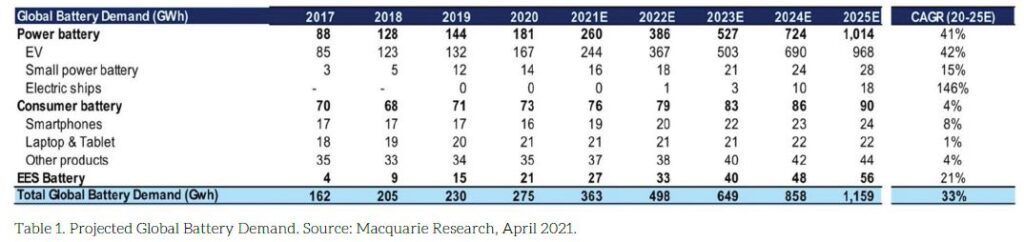
At the moment, Europe does not have any refining capacity to do this. This is concerning as most of the world’s refining of lithium today takes place in China (with the Russian Federation as a distant second). Europe is very much exposed on the supply side and when looking at the global refining capacity it becomes clear that refining capacity will fall short drastically in the next two to three years (see Figure 1). It is therefore time that Europe starts to build its own capacity. Northvolt in Sweden is a nice example and will probably be Europe’s first battery ecosystem to serve the European market. Many more projects like Northvolt are needed to meet the demand that’s coming within the next decade.4
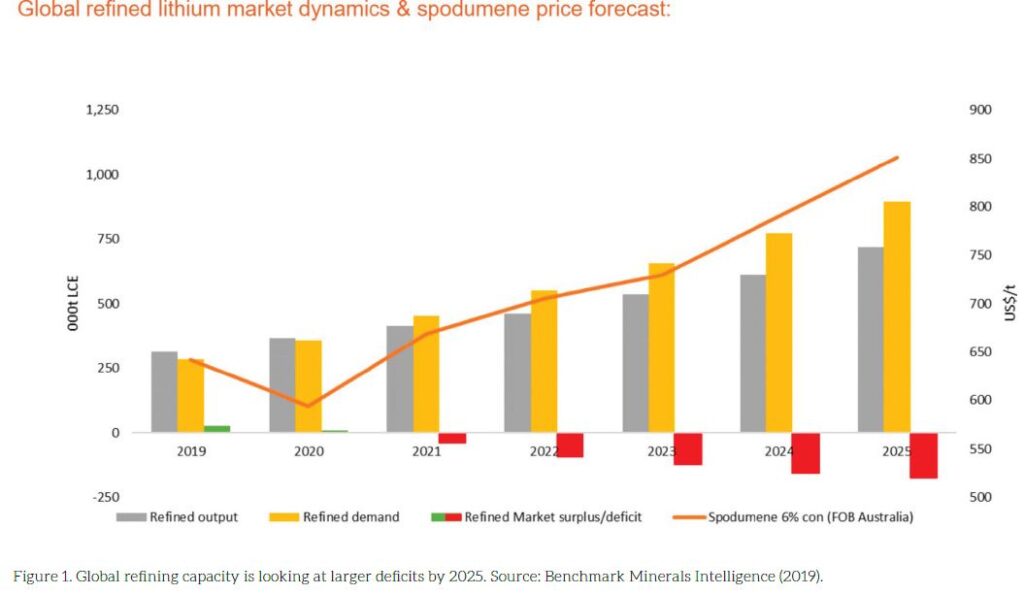
Nickel
Automotive electrification is set to become the single-largest contributor to the growing demand for nickel in the next decade. Nickel has become a very important mineral for EVs due to the fact that EV makers prefer batteries with a higher energy density as this increases the power and range of the EV. Under the current battery technology, nickel and cobalt are the critical elements to keep high energy density batteries stable. As more battery producers and EV makers try to thrift the use of cobalt by replacing it with nickel or manganese, nickel’s role in the energy storage sector is set to become more significant in the next decade. As with all other critical minerals, nickel cannot simply be extracted from ore and put into an end application. For lithium-ion batteries, nickel needs to be processed into nickel sulphate which is a high purity chemical compound.
Today, producing nickel sulphate has its challenges. First, there is only a very limited number of feedstock types that is suited for its production. These include Class 1 nickel and certain intermediate nickel products such as nickel matte, Mixed Sulphate Precipitate (MSP) or Mixed Hydroxide Product (MHP).5 Second, producing nickel sulphate from suitable intermediates often requires a processing method called High Pressure Acid Leaching (HPAL) which takes a toll on environment and communities as we have seen in Indonesia.6
Despite the recent announcement of the world’s biggest nickel producer Tsingshan that it has successfully produced nickel matte from Nickel Pig Iron (NPI), a Class 2 nickel type, normally not suitable as feedstock for batteries, it remains to be seen how disruptive Tsingshan’s process will be. First, it seems as if Tsingshan’s new process requires a lot more energy which will not benefit the ESG footprint of the product. Second, producing nickel sulphate from Class 1 nickel often comes with a nice by-product: cobalt. Having cobalt as a by-product improves the economics for nickel producers. Third and finally, the iron content in NPI can negatively impact the purity levels of the nickel sulphate output from Class 2 extraction. Cathode manufacturers tolerate only extremely low iron contents in the nickel sulphate they purchase. Until these challenges are resolved Class 1 and nickel intermediates remain the most important sources to feed the EV supply chain, posing some real challenges for the production of batteries and EVs.
Cobalt
Cobalt is widely used in alloys to make (engine) parts that require strength and durability at high temperatures. These alloys are also known as super alloys and are used in aerospace (jet engines) and the defence industry (rockets). Traditionally, super alloys have been the most important application for cobalt. However, over the past 20 years a new market has increased in significance for cobalt demand: batteries. From smartphones to high-end EVs, the batteries that power these machines have chemistries in which cobalt plays a prominent role as a stabilizer. Cobalt allows cathode makers to apply chemistries that increase the energy density of the battery without worrying too much about its chemical stability. The expectation is that by the end of the decade EV batteries will have become the primary application for cobalt with an annual demand for the mineral of at least 120,000 tonnes per year, an increase of more than 300% compared to the demand coming from EVs today.7 However, a couple of factors limit the supply and use of cobalt. First, almost all cobalt produced today originates as a by-product from copper and nickel production, as illustrated in the simplified flowsheet in Figure 3. It takes hydrometallurgical and pyro metallurgical processes to separate cobalt from the nickel or copper produced, an energy intensive and ecologically draining production method. Second, as with lithium and nickel, cobalt needs further processing before it can be put into end-applications. Cobalt sulphate is the chemical used in cobalt-based batteries. China is the main producer of cobalt sulphate today. With 70% of the global cobalt refining capacity on its territory, China plays a pivotal role in the cobalt supply chain. And it will likely continue to do so in the next decade because the competition (Finland and Belgium in the EU and Canada in North America) is unlikely to catch up before the end of this decade. Which dovetails into the third and final limiting factor: the limited alternatives to today’s suppliers. Today, the Democratic Republic of Congo (DRC) is by far the largest producer of cobalt and China is by far the largest exporter of cobalt sulphate. Although these countries play a critical role in the cobalt supply chain, they also have a complicated relationship with the EU. Combined with the concerns raised by human rights organizations around the poor working conditions in some of the smaller mines in the DRC, the appeal to thrift the use of cobalt is becoming stronger.
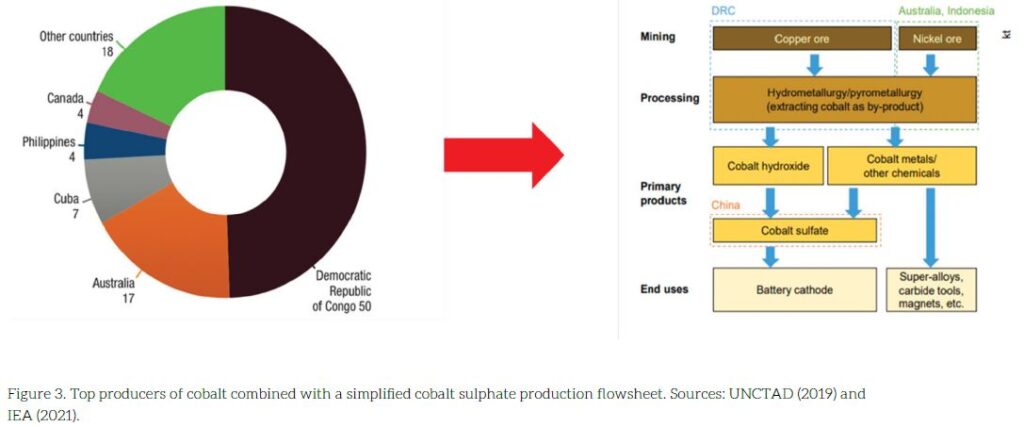
Copper
World copper consumption is projected to grow at an average 2.5% per year to reach almost 28 million tonnes by 2025 (see Table 2 below). Copper’s high conductivity and durability gives it a crucial role in many end applications. Although end-applications like EVs, lithium-ion batteries and renewable energy systems are becoming more important, the largest demand for copper today derives from electricity networks and applications in construction.8 Demand from electricity networks is expected to get a boost as the need for fast charging stations will increase exponentially with more EVs coming on the roads. Fast chargers require thick, high amperage cables with copper being the preferred conductor. Currently, China consumes half of the world’s copper. China’s 14th Five Year Plan announced accelerated investments in high-speed rail, telecommunications, electrification of transport and renewable energy solutions like solar PV plants and wind parks.9 All these end-applications need copper and lots of it. And with the trillion-dollar infrastructure plan announced by the Biden administration, demand from the United States is expected to increase as well. China’s plan and the ambitions of President Biden can potentially create a very tight market in the coming years. Looking at Table 2, two things become clear: copper consumption has overtaken copper production from mines and refineries, explaining the recent price hikes for the metal.10 Second, copper stockpiles will decrease in the coming years and by 2026 will reach volumes just enough to meet global consumption for only 1.7 weeks. Low stockpiles are a recipe for disaster. As we have seen in other markets (like LNG), having enough stock capacity is crucial to mitigate unexpected market disruptions. At the same time there are a couple of structural challenges that the industry will have to overcome to significantly increase the copper production in the mid to long term. First, mine operators face declining ore grades. This might not be an issue in a high price environment but since mining projects are long term projects, prices would need to remain high (enough) to justify the higher cost of extracting smaller volumes of copper. Second, commer- cially viable copper deposits are geographically scarce. Currently, roughly 40% of primary copper production comes from Chili and Peru and this is likely to remain that way in the coming decade.11
In addition, it’s becoming increasingly more expensive to produce primary copper. The 20 or so projects that are under development in the world have a combined CAPEX of US$65 billion. The expected output from these projects is 3.3 million tonnes of additional primary production. Put that in the context of 2020 annual demand for copper (just over 25 million tonnes, see Table 2) and it becomes clear why the market is so nervous at the moment.
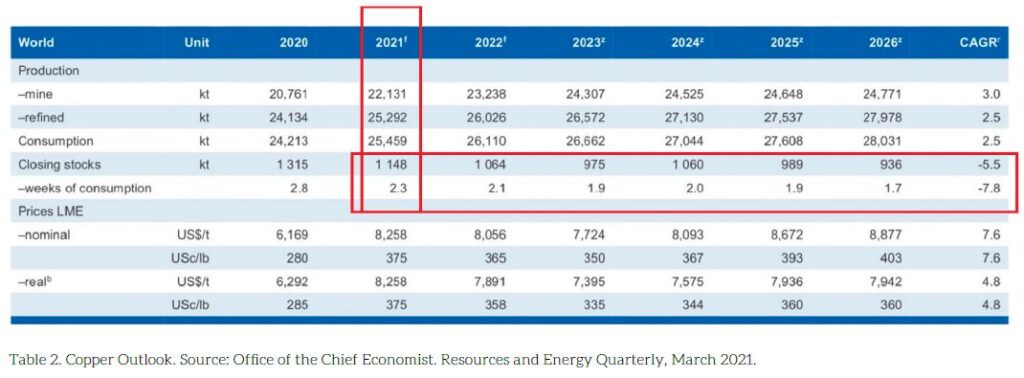
Graphite
Graphite has two characteristics that make it useful for a diverse set of end-applications. First, the honeycomb-like structure makes graphite flexible but strong at the same time. Second, the many layers of which graphite is made of offer a perfect spot to catch and release lithium ions that travel back and forth between a battery’s positive electrode (i.e., the cathode) and a battery’s negative electrode (the anode) whilst the battery charges and discharges. The first characteristic makes graphite very important in the manufacturing of steel, the second characteristic makes graphite the mineral of choice for lithium-ion batteries and their end applications. As illustrated in the section on lithium demand, LiB capacity is set to grow to 1 TWh by 2025. Unless some superior technology becomes scalable before 2025, all these batteries will need graphite for their anodes. Although a deficit in the supply of natural graphite is not expected in the next few years, there are a couple of important factors that will affect the supply of graphite to batteries.
There are five different graphite types currently produced in the world (see Figure 4 for an overview of the different graphite types). Today, the two primary graphite types used for lithi- um-ion batteries are: flake graphite and synthetic graphite. China is the world’s biggest producer of synthetic graphite. Synthetic graphite is produced from by-products of oil refining (petroleum cokes for instance). The production of synthetic graphite is energy intensive, eco-unfriendly, lengthy, and capital intensive. Anode makers are using synthetic graphite because it has long held an edge over flake graphite by delivering superior performance in the anode.
While China is the world’s biggest producer of synthetic graphite, it is also the world’s biggest consumer of it as the world’s largest battery manufacturers are located in China. This will put a strain on the export of synthetic graphite which, due to its bad ESG footprint, also will have to deal with the scrutiny of EU environmental regulation.
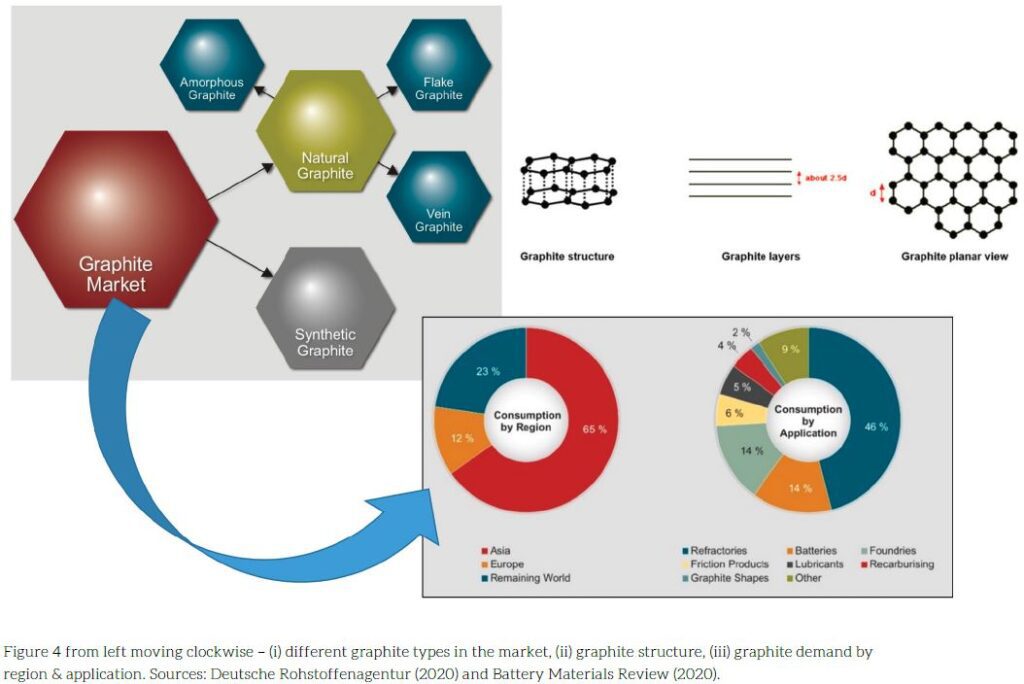
Given the fact that the performance of flake graphite in anodes has improved over the recent years and the fact that the production of flake graphite is less capital intensive, quicker and cleaner than the production of synthetic graphite it is expected that there will be increased investments in natural (flake) graphite projects outside of China over the coming years. It then becomes very important for the EU to secure a foothold in these projects, and get involved in the development of these projects. This way, the EU will get graphite into its supply chains which is crucial if it wants to meet its ambitions with lithium-ion batteries. But securing access to natural graphite also means that the EU can secure enough natural graphite to meet demand in the other sectors that are of strategic importance such as steel production.
Manganese
As mentioned in the section on nickel, EV makers are actively seeking ways to increase the use of nickel and manganese in the cathode chemistries of lithium-ion batteries. High Purity Electrolytic Manganese Sulphate Monohydrate (HPMSM) and High Purity Electrolytic Manganese Metal (HPEMM) are used as feedstock for the type of batteries that Europe wants to use in high performing EVs. However, only 2% of global EMM production in 2019 was high purity with no selenium.12 Selenium dioxide is added to manganese to remove impurities and helps to reduce the cost of refining manganese. However, selenium is harmful to the environment and is not suitable for manganese sulphate used in batteries. Selenium-free EMM is produced in China and South Africa. Manganese Metal Company, in South Africa, is the largest refiner of high purity selenium-free manganese with a production capacity of about 30,000t. Euro Manganese Inc. is building Europe’s only selenium-free EMM refining facility in the Czech Republic, targeting the HPMSM market. The shift away from cobalt dominated cathodes will create an increased demand for both HPEMM and HPMSM. Here is the issue though: HPEMM and HPMSM have a limited feedstock. Today, it is only economically viable to extract high purity manganese from ores containing manganese carbonate. Only 2% of all manganese holding orebodies in the world are manganese carbonate ores. And there is literally only a handful of projects outside of China that have the potential to produce manganese carbonate.13 With just one manganese refining project within its border the EU is dependent on the available refining capacity in countries like China.
Rare Earth Elements (REE)
Although it is common to refer to rare-earth elements (REEs) as one group of critical minerals, it is worth clarifying that REEs comprise of 17 different elements which can be classified in two types, depending on each element’s weight and atomic number:
- Mid- to heavy REEs (MHREEs). These REEs appear in lower volumes per ton of rare-earth deposits, thereby making them more difficult to exploit economically. MHREEs are commonly used in glass and laser optics, metals and alloys, and semiconductors.
- Light rare earth (LREEs). LREEs are mainly used in permanent magnets and electronics, with neodymium being especially important for the production of permanent magnets.
Currently, permanent magnets are the biggest drivers behind the demand in REEs and this demand from permanent magnets manufacturers is set to increase over the next decades as illustrated in Figure 5 above. Today, it is China that dominates the production of permanent magnets. About 90% of today’s supply is controlled by China.14
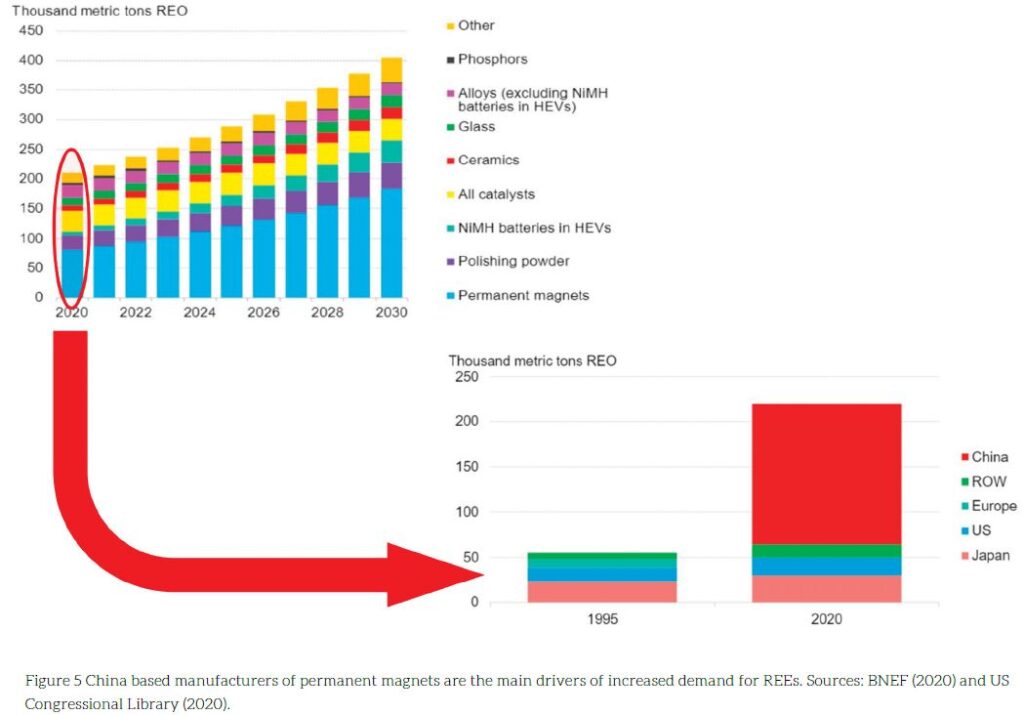
Permanent magnets improve the performance of generators and electric motors. Both in terms of increased energy density and operational longevity. This last point is notably impor- tant for offshore wind farms as these sites are located at more distant sites and operate under tougher conditions. Having low maintenance and efficient generators keeps operating expenditures (OPEX) under control.15 Wind turbines and EVs are two important drivers behind the demand for permanent magnets and given the crucial role these two-end applications play in the transition to a cleaner energy system as illustrated earlier in Figure 2, it should not come as a surprise that the demand for permanent magnets will rise this decade. And, at least until there is a viable alternative to them, REEs will remain in the spotlight of manufacturers of wind turbines and EV makers.
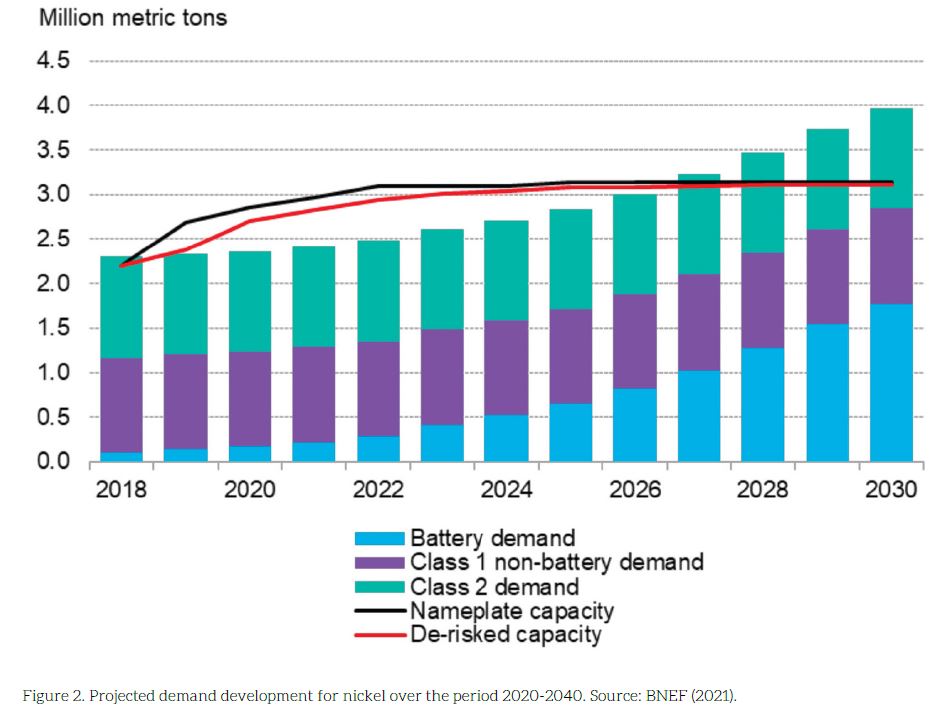
But producing the REEs that are required to manufacture permanent magnets is not an easy thing. REEs occur in ores as a group, clustered together containing both LREEs and MHREEs. Some REEs, particularly MHREEs, occur in lower concentrations in deposits than others.
For example, to produce enough dysprosium and neodymium (two REEs that are very important for the production of permanent magnets), miners end up producing more of other, less critical elements like lanthanum and cerium as well. Much more in fact than needed. This over-supply pushes down the price of lanthanum and cerium even further. In the meantime, producers have to average the price and costs across the basket of REEs they produce for every ton of ore processed. If prices for lanthanum and cerium are too low, producers will not be incentivized to produce more volumes of REEs to obtain elements like neodymium and dysprosium that are in high demand. This phenomenon is referred to by the industry as “the balance problem” and it has led to an oversupply of lanthanum and cerium in 2016. Since then, the industry has started to look for ways to solve the balance problem as it can seriously threaten the supply of the more valuable elements that are required for permanent magnets. Possible solutions that are explored today include: increasing the application options of less valuable REEs (i.e., look for new ways to make lanthanum useful) and increasing R&D efforts to use less or no REEs altogether. Although this last point seems like a “no-brainer” one has to keep a couple of things in mind.
First, it takes time to find or develop a substitute. Second, creating a technically viable substitute that can be produced in a scalable manner outside of a laboratory is equally challenging. Finally, and this is especially true for offshore wind projects, it is important to realize that a lot of planning, thought, calculations and financial modelling is put into the development of an offshore wind project. The planning and purchasing of crucial parts of a project is done years before a project is ready for commercial operation.
Changing turbine types is not something that can be done overnight. Therefore, it is very likely that most of the projects for offshore wind generation that are in the pipeline today will still use turbines with direct-drive generators that contain permanent magnets as essential parts of their operation, even if there is a substitute for REEs tomorrow.
1 One TWh equals 1 billion kWh in energy storage capacity.
2 Lithium Carbonate Equivalent or LCE is the collective name for lithium chemical compounds used in cathodes. The rule of thumb used in the industry is that a typical EV requires 0.7 to 0.8 kg of LCE for 1 kWh of energy storage capacity.
3 Our ‘back on the envelope’ calculation assumed a 50 kWh batter pack for each EV sold in 2030. 10 million times 50kWh makes 500 million KWh which equals 500 Gigawatthours (GWh).
4 See: https://en.wikipedia.org/wiki/Northvolt
5 Nickel products can be classified into two categories: Class 1 nickel which meets a purity standard of at least 99.8% of nickel metal, and Class 2 nickel which has a purity level of less then 99.8%.
6 HPAL stands for High Pressure Acid Leaching. As the name suggests, it’s a process that requires two things that governments want to reduce: energy (and lots of it) to create high pressure and acids which leave a mark on communities and the environment in the form of hazardous tailings disposals. Indonesia recently banned deep sea tailing disposals.
7 Global Cobalt Outlook 2021-2030, BNEF 24 May 2021.
8 Office of the Chief Economist. Resources and Energy Quarterly. March 2021.
9 Ibid.
10 Reference to LME and spot prices, see Office of Chief Economist p.124
11 Benchmark Minerals Intelligence, Gianni Kovacevic. Q1 review 2021.
12 Global Manganese Outlook 202o-2030, BNEF 18 December 2020.
13 Fernley, Matt, Some Manganese is more equal than others, Battery Minerals Review, 2 November 2020
.14 See: https://hcss.nl/report/securing-critical-materials-for-critical-sectors-policy-options-for-the-netherlands-and-the-european-union/ and https://www.reuters.com/business/autos-transportation/china-frictions-steer-electric-automakers-away-rare-earth-magnets-2021-07-19/
15 ‘Rare Earth Demand in Clean Energy’, BNEF 14 September 2020.